The search for a superconductor that can work under less extreme conditions than hundreds of degrees below zero or at pressures like those near the center of the Earth is a quest for a revolutionary new power – one that’s needed for magnetically levitating cars and ultra-efficient power grids of the future.
But developing this kind of “room temperature” superconductor is a feat science has yet to achieve.
A University of Central Florida researcher, however, is working to move this goal closer to realization, with some of his latest research published recently in the journal Communications Physics – Nature.
In the study, Yasuyuki Nakajima, an assistant professor in UCF’s Department of Physics, and co-authors showed they could get a closer look at what is happening in “strange” metals.
These “strange” metals are special materials that show unusual temperature behavior in electrical resistance. The “strange” metallic behavior is found in many high-temperature superconductors when they are not in a superconducting state, which makes them useful to scientists studying how certain metals become high-temperature superconductors.
This work is important because insight into the quantum behavior of electrons in the “strange” metallic phase could allow researchers to understand a mechanism for superconductivity at higher temperatures.
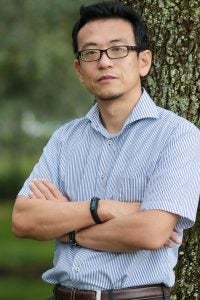
“If we know the theory to describe these behaviors, we may be able to design high-temperature superconductors,” Nakajima says.
Superconductors get their name because they are the ultimate conductors of electricity. Unlike a conductor, they have zero resistance, which, like an electronic “friction,” causes electricity to lose power as it flows through a conductor like copper or gold wire.
This makes superconductors a dream material for supplying power to cities as the energy saved by using resistance-free wire would be huge.
Powerful superconductors also can levitate heavy magnets, paving the way for practical and affordable magnetically levitating cars, trains and more.
To turn a conductor into a superconductor, the metal material must be cooled to an extremely low temperature to lose all electrical resistance, an abrupt process that physics has yet to develop a fully comprehensive theory to explain.
These critical temperatures at which the switch is made are often in the range of -220 to -480 degrees Fahrenheit and typically involve an expensive and cumbersome cooling system using liquid nitrogen or helium.
Some researchers have achieved superconductors that work at about 59 degrees Fahrenheit, but it was also at a pressure of more than 2 million times of that at the Earth’s surface.
In the study, Nakajima and the researchers were able to measure and characterize electron behavior in a “strange” metallic state of non-superconducting material, an iron pnictide alloy, near a quantum critical point at which electrons switch from having predictable, individual behavior to moving collectively in quantum-mechanical fluctuations that are challenging for scientists to describe theoretically.
The researchers were able to measure and describe the electron behavior by using a unique metal mix in which nickel and cobalt were substituted for iron in a process called doping, thus creating an iron pnictide alloy that didn’t superconduct down to -459.63 degrees Fahrenheit, far below the point at which a conductor would typically become a superconductor.
“We used an alloy, a relative compound of high temperature iron-based superconductor, in which the ratio of the constituents, iron, cobalt and nickel in this case, is fine-tuned so that there’s no superconductivity even near absolute zero,” Nakajima says. “This allows us to access the critical point at which quantum fluctuations govern the behavior of the electrons and study how they behave in the compound.”
They found the behavior of the electrons was not described by any known theoretical predictions, but that the scattering rate at which the electrons were transported across the material can be associated with what’s known as the Planckian dissipation, the quantum speed limit on how fast matter can transport energy.
“The quantum critical behavior we observed is quite unusual and completely differs from the theories and experiments for known quantum critical materials,” Nakajima says. “The next step is to map the doping-phase diagram in this iron pnictide alloy system.”
“The ultimate goal is to design higher temperature superconductors,” he says. “If we can do that, we can use them for magnetic resonance imaging scans, magnetic levitation, power grids, and more, with low costs.”
Unlocking ways to predict the resistance behavior of “strange” metals would not only improve superconductor development but also inform theories behind other quantum-level phenomena, Nakajima says.
“Recent theoretical developments show surprising connections between black holes, gravity and quantum information theory through the Planckian dissipation,” he says. “Hence, the research of ‘strange’ metallic behavior has also become a hot topic in this context.”
Co-authors included researchers from the University of Maryland; the National Institute of Standards and Technology Center for Neutron Research; the National High Magnetic Field Laboratory at Florida State University; the Leibniz Institute for Solid State and Materials Research in Dresden, Germany; the Shanghai Institute of Microsystem and Information Technology at the Chinese Academy of Sciences in China; and the Canadian Institute for Advanced Research in Toronto, Canada.
The research was funded by the National Science Foundation Division of Materials Research, the Gordon and Betty Moore Foundation’s EPiQS Initiative. Some of the work was performed at the National High Magnetic Field Laboratory, which is supported by an NSF cooperative agreement with the State of Florida. Pressure measurements were supported by the National Institute of Standards and Technology.
Nakajima received his doctorate in physics from the University of Tokyo in Japan and worked as a postdoctoral research associate at the Center for Nanophysics and Advanced Materials at the University of Maryland. He joined UCF’s Department of Physics, part of UCF’s College of Sciences, in 2016.